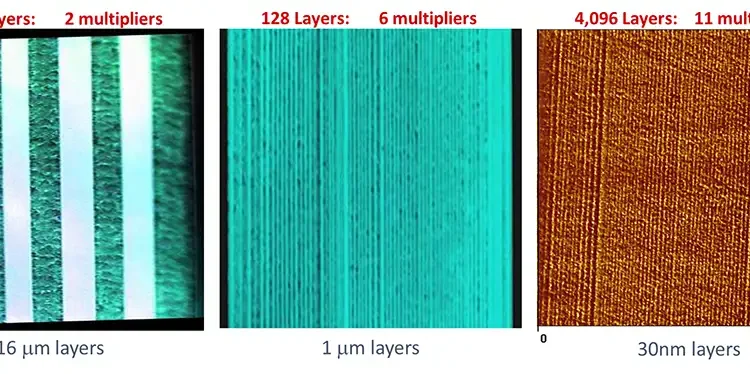
By Michael Ponting, Ph.D., co-founder & president, and Deepak Langhe, dir.-Energy Storage Technology, PolymerPlus LLC
Editor’s Note: This technical paper is based on a presentation at the 2019 AIMCAL R2R Conference USA / SPE FlexPackCon, held in Myrtle Beach, SC, in October 2019.
Abstract
Micro- and nanolayer coextrusion processing incorporates tens, hundreds or thousands of alternating polymer layers in the envelope of traditional packaging films. The reduction of the individual polymer-layer thicknesses down to 25- to 50-nanometer range, through this specialized form of cast coextrusion, has enabled processors to produce films with unusual optical reflections, enhanced oxygen and/or water-vapor barrier, and increased toughness. The following article describes the micro- and nanolayer coextrusion processing technology and the polymer-material selection criteria required for nanolayer uniformity. It also reviews polymer-structure, property-induced enhancements in layered-polymer film systems constructed of traditional polymer-packaging resins.
1. Introduction
Micro- and nanolayered films are comprised of two or more polymer materials highly ordered via coextrusion into parallel layered domains that range in individual-layer thicknesses from the single microns down to 10s of nanometers. As a result of these thin, individual-layer thicknesses, coextruded films’ internal layer counts increase from the standard 7-11 to 32 or more in micro- and nanolayered films. Increased development activity is possible due to more readily available commercial tooling suppliers and a growing base of demonstrated enhancements of film systems constructed from conventional, commercial polymer materials. To this end, micro- and nanolayered, coextruded cast-polymer films have been demonstrated at production scales ranging from laboratory and pilot line all the way to commercial-manufacturing systems processing films at 100s of mpm.
To highlight the technical performance advantage of micro- and nanolayered polymer films, this article first will review the cast coextrusion-processing approach and material-selection rules for coextrusion. Additionally, selected case studies in structure-property performance of micro- and nanolayered material systems will be highlighted for optical, packaging and specialty film applications.
2. Micro- and nanolayer coextrusion process
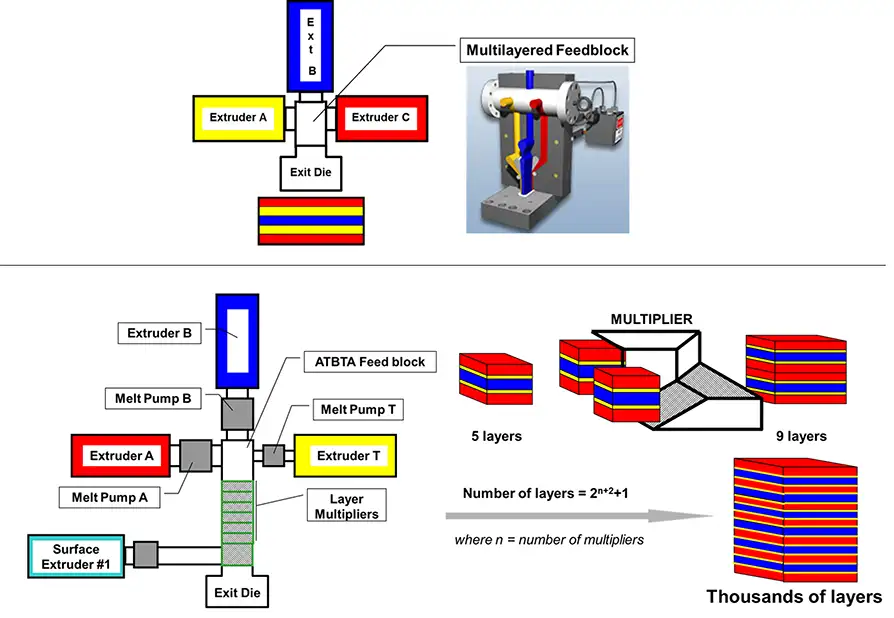
The combination of two or more polymer materials to enhance the overall composite properties has been studied extensively using various traditional processing techniques, including melt blending [1], block copolymerization [2,3] and multilayer coextrusion [4-7]. Micro- and nanolayering coextrusion is an advanced cast- or blown-film processing technique capable of continuous film coextrusion with tens to thousands of individual layers. Production of films with several thousand layers results in the reduction of individual-layer thicknesses from the micron-scale down to as low as 25 nanometers.
Nanolayer film production enables an opportunity to magnify the concentration of interfacial or confinement-induced phenomena as they become dominant with a reduction of individual-layer thicknesses toward the macromolecular dimensions of the individual polymer materials [4,5]. The uniqueness of the nanolayered film-production process is in the combination of conventional coextrusion extruders with either a microlayered feed block [6] and/or with the addition of a series of sequential-layer multiplication dies [7] (see Figure 1). The sequential-layer multiplier design case creates a highly flexible coextrusion process for producing polymeric films with tens to thousands of layers with simpler tooling changes for development and pilot lines. The micro- and nanolayered, single feed block approach is better suited for production environments where a material system, formulation and number of layers is known and higher production rates allow processing in large batch trials. The single feed block approach for instantaneous coextrusion of micro- and nanolayered structures also results in a higher individual-layer thickness uniformity (1-5% variation of layer thickness) as compared to the sequential-layer multiplication approach (5-15% layer thickness variation).
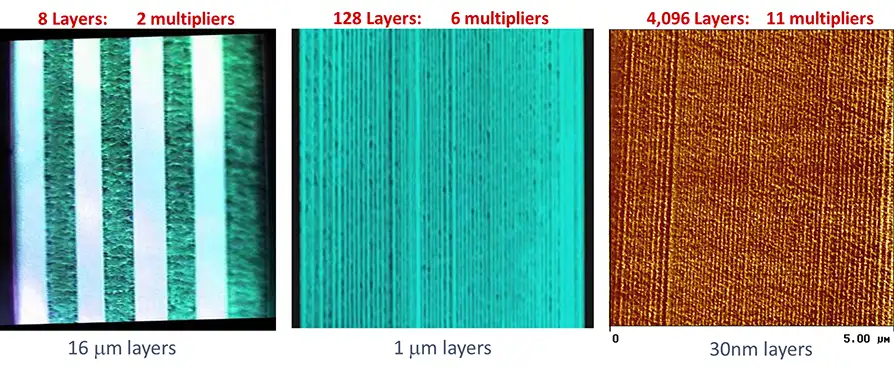
A series of coextruded cast-layered films with increasing numbers of layers is shown in Figure 2. The figure displays microscope cross-section images of cast-layered film after cryo-microtoming. The 8- and 128-layer films were imaged using an optical micrograph, while the 4,096-layer film was imaged via an atomic force microscope (AFM). All films were polymethylmethacrylate (PMMA)/polycarbonate (PC) multilayer films produced via nanolayer coextrusion via the sequential-layer multiplication approach shown in Figure 1.
2.1 Blown-film coextrusion for packaging applications: Though only previously discussed for cast-film processing, blown-film coextrusion is extensively used to fabricate packaging film, much of which is multilayered to improve mechanical, transport, thermal properties as required by the food or medical industry. Research efforts, simultaneously conducted at The Dow Chemical Co. and Cryovac/Sealed Air Corp. [8], have resulted in process-technology advances to enable micro- and nanolayer coextrusion capabilities for blown-film processing lines. Development of blown-film coextrusion, multilayered feed blocks with greater than 20 layers, though not yet at thousands, and sequential-layer multiplication dies have been reported following the historical development path of cast-film coextrusion. Currently, multilayer-film lines are commercially available from many equipment manufacturers at varying numbers of layers including, but not limited to, Davis Standard, Macro Engineering, Alpha Marathon, Bandera and Windsor.
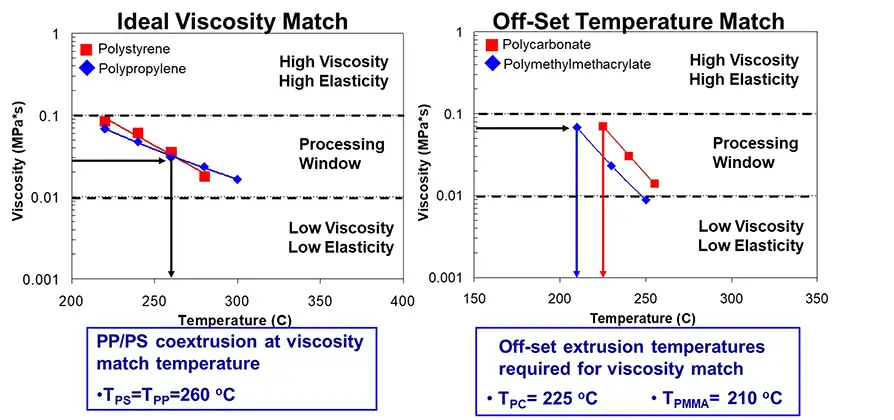
2.2 Viscosity requirement for coextrusion: When selecting polymer materials and formulations for micro- and nanolayer-film coextrusion trials, the relative viscosity behavior of the constituent polymer materials is a critical parameter to achieve layer uniformity in the final film. Polymer-material candidates for microlayer-coextrusion processing should be selected with a similar viscosity near the desired polymer-material, coextrusion-processing temperature. When measuring and comparing the material rheological behaviour, selection of process-relevant shear rates is required. Though polymer materials are sheared and melted at a frequency greater than
100 s-1 in extrusion barrels and screws, the layering process occurs at a relatively lower-shear region (10-100 s-1 in the multilayer feed block and/or layer-multiplication flow channels.) A temperature dependent rheological measurement of polymer materials over this shear range and potential processing temperatures always should be examined prior to processing to ensure a viscosity overlap or near-match (see Figure 3) to maintain layer parallelism and continuity in the final produced material.
Though a precise threshold for how mismatched the polymer-material rheology can vary is not fully understood, a first approximation limit of no more than (a) a 15° C difference in processing temperature and (b) a 1.5X difference in the relative material viscosity at the feed block or multiplier shear rate is recommended for processing trials. If rheological properties of the materials are not considered and matched prior to processing, the potential for a poor viscosity match can result in the lower-viscosity polymer encapsulating and/or forming a slip film between the higher-viscosity polymer and the multiplier-die wall [9,10]. Interfacially driven layer instability and breakup also may result during layer multiplication if the two polymer melt-streams possess a relatively large viscosity mismatch [11].
3. Novel properties of micro- and nanolayered polymer films
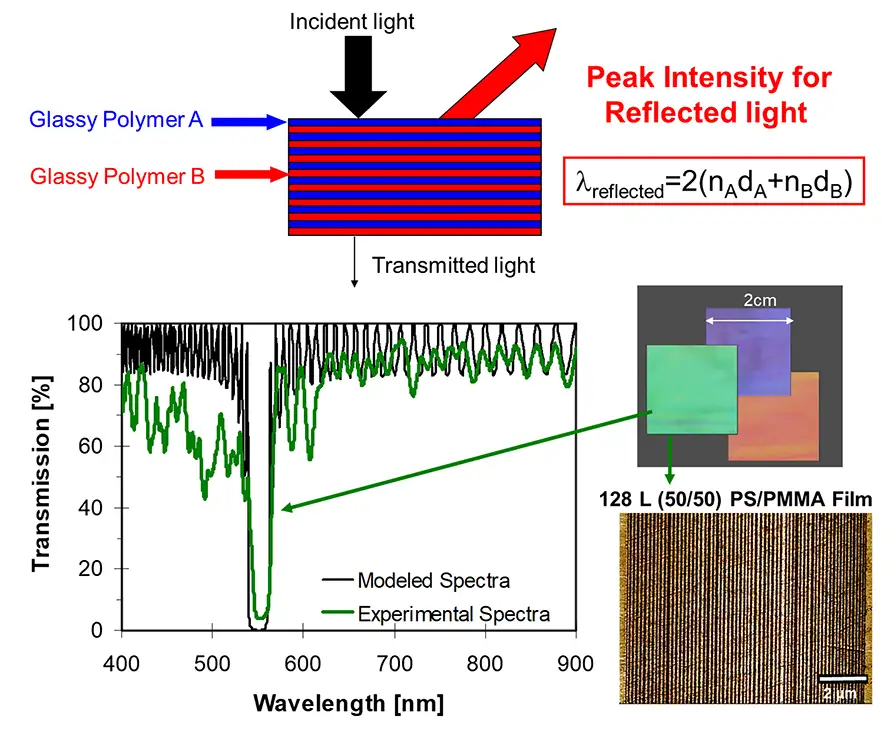
3.1 First commercial applications: optically reflective films: The first realization of a commercial application of nanolayered film was published in the early 1960s. The first polymer films with tens or hundreds of layers were processed via a system of sequential-layer multiplying dies with two alternating glassy, amorphous polymers. The resulting layered films displayed bold, structurally induced optical reflections and physical coloring similar to that of the phenomena seen in butterfly wings in nature. These early nanolayered films, reported by Alfrey, et al. [12], were comprised of 201 repeating layers of two different polymer materials that possessed a significantly different refractive index (Δn= 0.1). Alfrey translated relationships previously used in inorganic, vapor-deposited Bragg crystals or “soap bubble in air” periodic layered systems to describe the phenomena via a mathematical model so as to predict and formulate the optical appearance and color of the nanolayer films (see Figure 4). The model used polymer-material, refractive-index information and layer thicknesses to calculate optical reflection peaks due to interfacial reflections within the films.
The first applications leveraging the unusual optical reflection of coextruded micro- and nanolayered, polymeric Bragg crystal films were nontechnical, consumer-decorative applications. The technology was first licensed for production of decorative, optically reflective films [13-15] including gift paper, prismatic bags and top-coat films for lamination molding in the 1970s. Eventually, an additional sublicense for the optical micro- and nanolayered film was completed in the 1990s, leading to the technology eventually finding applications as a component in electronic monitors and displays as a polarizing and brightness enhancing film. Today, polymeric micro- and nanolayered Bragg films are produced for architectural window coverings and thermal reflectors via a similar approach [16]; however, the optical reflective bands for the material reside in the infrared wavelengths.
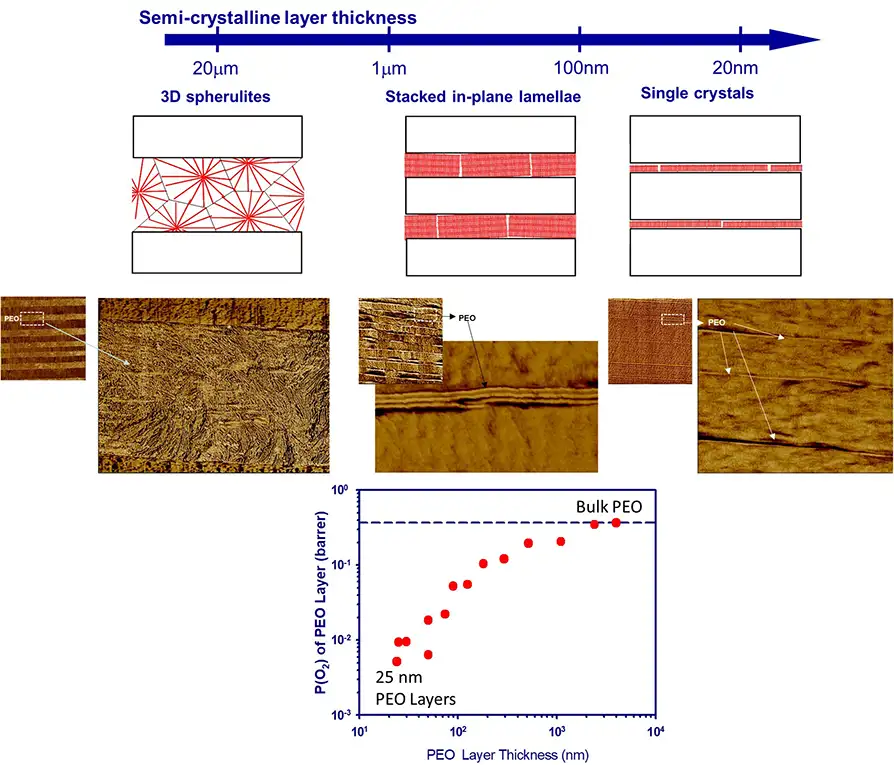
3.2. Current development thrust: Enhanced barrier for consumer packaging: The micro- and nanolayer coextrusion approach to producing high oxygen- and water-vapor-barrier films offers an additional opportunity to shift polymer-material morphology as a result of nanoconfinement in very thin layers. Nanolayered-film coextrusion processing [17] allows production of multilayer films with thousands of layers with each layer thickness approaching the size scale of the radius of gyration of polymer-material chains. Restriction of semi-crystalline polymer materials into these highly confined, nanolayered spaces has resulted in a change of polymer-crystallization behavior from a 3D space filling crystal spherulite, into two-dimensional, high aspect-ratio parallel platelets in the plane of the nanolayers (see Figure 5). This change in material-crystallization behavior has led to a large drop, greater than 100X, for oxygen transport in polymers such as polyethylene oxide and polycaprolactone, and resulted in these conventional, commercial polymer materials achieving gas barrier approaching barrier-grade Nylon material properties.
The mechanism for the reduction in gas permeation through the confined, layered polymer film can be attributed to an increase in tortuosity of gas molecules diffusing through the film. The intralayer crystalline phases of polymers, now shaped in high aspect-ratio stacks of platelets oriented normally to the transport direction of the gas molecules, are typically considered impermeable to the diffusing permeant. This restricts molecular-gas transport to mainly around the crystalline domains in the polymer-layer, amorphous-phase gaps. The amount of crystallinity and lamellar orientation can be controlled by varying the layer thickness in the nanolayered films and controlling the size and spacing of these gaps to reduce gas permeation through the nanolayered polymer film.
Published efforts have reported confinement of PEO lamellar in 25-nm-thick layers to result in platelet-crystal growths with an aspect ratio much greater than 100 that suggest an effective addition of micron-area barrier blocking crystals in the layered polymer film due to the confined crystallization approach [18]. This phenomena of layer confinement for increased oxygen or water barrier has been experimentally demonstrated in several additional, semi-crystalline polymer materials, including polycaprolactone, polyvinylidene fluoride, high-density polyethylene, syndiotactic polypropylene and poly 4-methylpentene-1. Potential inclusion of this nanolayering-induced, barrier-enhancing mechanism also has been adapted into biaxially oriented polypropylene and polyester films through inclusion of 10-20% of the previously described polymer materials [19] for use in medical, food, electronic and consumer-goods applications.
3.3. In-development application: Energy-storage films: Advanced solid-state, electronic energy-storage capacitors have been demonstrated from films combining nanolayers of high electric breakdown strength and high dielectric energy storage polymer materials [20]. A synergistic increase in the electrical breakdown strength of nanolayered polymer films resulted from delocalization of electrical charge across multiple film-interface layers that enable processing of films with energy densities up to 13.4 J/cm3. The nanolayer polymer-films structure enables the increase in polymer-film electrical performance through a combination of controlling electrical-damage propagation at the layer boundaries, a decrease in defect concentrations throughout the film that act as premature electric breakdown failure points and reduced electrical losses of the polymers as layers become thinner due to crystallization confinement of the nanoscale.
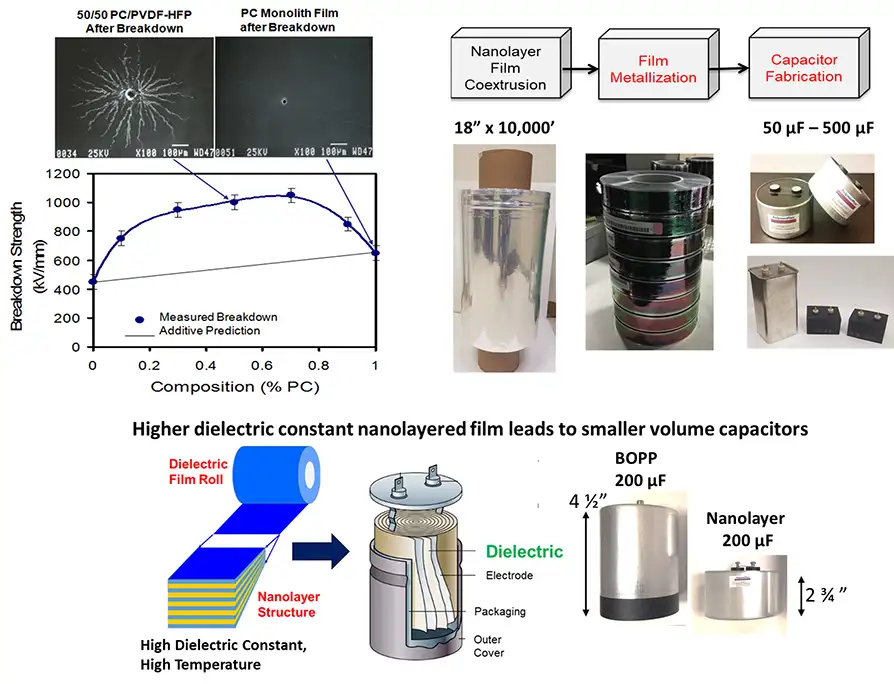
Electrical breakdown properties of 12-µm nanolayered polymer films were characterized on 32-layer polycarbonate (PC)/co polyvinylidene flouride-hexfluoropropylene (PVDF-HFP) films at relative composition intervals from 0/100 to 100/0 (see Figure 6). PC was chosen based on its excellent insulating properties and high breakdown strength (Eb=850kV/mm) while PVDF-HFP was used for its high dielectric constant (εr=12). The dielectric breakdown strength of the nanolayered films was determined under quasi-homogeneous field conditions using a sphere-plane geometry at a ramp rate of 500 V/s, as previously described [20].
The nanolayered PC/PVDF-HFP films exhibited enhanced breakdown strength, compared to the neat polymers, at all compositions tested. Due to the synergies previously discussed from the layer confinement and interfacial boundary contributions, the resulting nanolayer-film energy density as high as 13.4 J/cm3 with a low loss factor (tan δ) was demonstrated for a 70/30 PC/PVDF-HFP film sample. A mechanism for the enhanced electrical properties of the nanolayered films was related to the delocalization of the film input charge across the nanolayer boundaries in the plane of the film to spread the local breakdown occurrences in the film and maintain electrical utility. The 250% increase in the electrical breakdown of the nanolayered films over the neat polymer-control films was attributed to this delamination and failure indicative of the nanolayered film breakdown.
Current conversation of these nanolayered materials into higher energy density, high operating temperature (140° C vs. current 85° C BOPP state-of-the-art) capacitors is in progress with initial prototype data for 250-µF-sized capacitor work funding by the Office of Naval Research and the US Dept. of Energy. Target application space for these higher-performance capacitors include pulsed power, electric vehicle and high-temperature applications. An additional advantage of increasing nanolayered PC/PVDF energy density to ~5-13 J/cm3 over the current 2-3 J/cm3 of the BOPP state of the art is a potential reduction in film-capacitor volume by a factor of 2X or greater as the nanolayered-film thickness is reduced to match that of the current BOPP and BOPET parts.
Conclusions
Micro- and nanolayered coextrusion is a 50-year-old technique gaining traction in commercial film production. Reflective optical properties distinct to the nanolayered structure are commercialized. New discoveries, including nanolayer confined semi-crystalline polymers for food and medical packaging and high-temperature, energy-storage film capacitors, are examples of higher value-added properties in nanolayered films.
Acknowledgements
Research was supported by the NSF Science and Technology Grant DMR-0423914. Dielectrics technology work was supported through ONR award no. N00014-12-M-0096 and DOE EERE award no. DE-EE0007211. Layering processing studies supported through ARL co-operative award W911NF-17-2-0080.
References
1. Paul, D.R. and Bucknall, C.B., Eds. Polymer Blends, Vol. 1. Wiley 2000.
2. Hamely, I.W., Fairclough, J.P., Terrill, N., Ryan, A., Lipic, P., Bates, F., and Towns-Andrews, E. Macromolecules. 29 (1996) 8835-8843.
3. Bates, F. and Fredickson, G.H. Annual Review of Physical Chemistry. 41 (1990) 525-557.
4. Liu, R.Y.F., Bernal-Lara, T.E., Hiltner, A., and Baer, E. Macromolecules. 37 (2004) 6972-6979.
5. Liu, R.Y.F., Bernal-Lara, T.E., Hiltner, A., and Baer, E. Macromolecules. 38 (2005) 4819-4827.
6. U.S Patent 6,905,324. “Interface Control,” Cloeren, 2005.
7. Ponting, M., Hiltner, A., and Baer, E. Polymer Nanostructures by Forced Assembly: Process, Stucture, and Properties. Macrmolecular Symposia. (2010) In-press.
8. US Patent 9,481,143. “Multilayered structures having annular profiles,” Dow Chemical Company, 2016. U.S. Patent Application 2011/0229701. “Multilayered active oxygen barrier film comprised of a plurality of microlayers,” Cryovac Inc., 2011.
9. Michaeli, W. Extrusion Dies for Plastics and Rubber: Design and Engineering Computations. Hanser Publishers (1992).
10. Dooley, J., Viscoelastic flow effects in multilayer polymer coextrusion, Ph.D. Thesis, 2002.
11. J. Zheng, T.P. Lodge, C.W. Macosko, J. Rheology. 50 (2006) 41-57.
12. Schrenk, W.J., and Alfrey Jr., T. In Polymer Blends Vol. 2. Academic Press (1978) 129-165.
13. US Patent 4,310,584. “Multilayered light reflecting film,” Mearl Corp., 1979.
14. US Patent 5,008,143. “Decorative objects with multi-color effects,” Mearl Corp., 1991.
15. US Patent 4,643,943. “Multi-layer polyolefin shrink film,” Cryovac LLC., 1985.
16. US Patent 7,632,568. “Solar control multilayer film,” 3M Innovative Properties Corp. 2005.
17. Wang, H., Keum, J.K., Hiltner, A., Baer, E., Freeman, B.D., Rozankski, A., and Galeski, A. Science 323 (2009) 757-760.
18. Ponting, M., Lin, Y., Keum, J.K., Hiltner, A., and Baer, E. Macromolecules. 43 (2010) 8619-8627.
19. Carr, J.M., Langhe, D.S., Ponting, M., Hiltner, A., and Baer, E. J. Materials Research. 27 (2012) 1326-1350.
20. Wolak, M.A., Pan, M.J., Wan, A., Shirk, J.S., Mackey, M., Hiltner, A., Baer, E., and Flandin, L. Appl. Phys. Letters. 92 (2008) 113301-3.
Michael Ponting, co-founder and president of PolymerPlus LLC (Valley View, OH), holds a Ph.D. in Chemical Engineering from Case Western Reserve University. He has served as the lead scientist and primary investigator during PolymerPlus’ 9+ years of operation while serving as a PI on several US DoE- funded, multilayered-materials technology development programs in optics and in broader applications of micro- and nanolayered systems. Ponting has more than 40 peer-reviewed publications, conference papers and five patents related to the production and structure-property relationships of multilayered polymer-film systems. He is a member of SPE and OSA (Optical Society of America). Ponting can be reached at 216-264-4818, mponting@polymerplus.net, www.polymerplus.net.